Wind-Borne Debris Impacts on Façades
Performance-Based Design to Enhance Building Envelope Resilience
Presented on October 9, 2024 at Facade Tectonics 2024 World Congress
Sign in and Register
Create an Account
Overview
Abstract
Climate change effects are causing an increase in extreme wind events’ frequency and severity, worldwide. Regions that previously were not prone to these extreme weather conditions, are increasingly searching for ways to strengthen urban resilience. In windstorm events, wind-borne debris is one of the major causes of building envelope damage. Wind-borne debris causes 40% of property damage in extreme wind events, being the major cause of façade damage during windstorms. Façades serve as the main defense for people and property from the external environment.
In the last 50 years, code and standard requirements have been created to provide wind-borne debris impact protection for the building envelope. International standards use standardized projectiles that are not always representative of local environments and objects that are recorded to fail and fly in windstorms.
An alternative performance-based design framework is presented, to identify case-specific impact test requirements, to mitigate the effects of wind-borne debris in extreme wind events. This performance-based approach aims to be used to verify the wind-borne debris resistance of façades. Building aerodynamics, the trajectory, and the velocity of specific debris elements are considered to deploy performance-based façade solutions.
A case study is presented to discuss how to identify case-specific façade impact test requirements. Typical roofing elements that are typically recorded to fail and fly during windstorms are analyzed through a flight trajectory analysis. Roof shingles are the debris elements considered in the case-study. The reference target building, the object of the design for this case study, is an essential facility, to avoid disruption of essential services, especially in the post-event scenario.
If new buildings and façade retrofit projects can improve their resilience to wind-borne debris impacts, there can be a notable mitigation of the overall consequences of extreme wind events. Adopting performance-based design impact tests, building envelope solutions can sustainably address local needs to improve urban resilience.
Authors
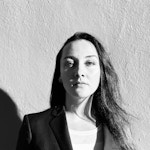
Angela Mejorin, Ph.D., Researcher
Founder and Curator
Performance-Based Façade Design
amejorin@gmail.com
Keywords
Paper content
INTRODUCTION
The evidence of climate change and its consequential increase in the frequency and intensity of extreme weather events is well-documented (IPCC 2022; Munich RE 2022). This trend is reflected
Access Restricted
Rights and Permissions
REFERENCES
Abdelhady, A.U., Spence, S.M.J., McCormick, J., 2022. Exogenous windborne debris: Definition and required extent of surrounding buildings for modeling in hurricanes. In: Journal of Engineering Structures 254 (2022) 11379874-76.
AS 1170.2:1989. Minimum design loads on structures, Part 2: Wind actions. Standards Australia.
AS/NZS 1170.2:2002. Structural design actions, Part 2: Wind actions. Standards Australia / Standards New Zealand.
AS/NZS 1170.2:2011. Structural design actions, Part 2: Wind actions. Standards Australia / Standards New Zealand.
AS/NZS 1170.2:2021. Structural design actions, Part 2: Wind actions. Standards Australia / Standards New Zealand.
ASCE 7-22, 2022. Minimum Design Loads and Associated Criteria for Buildings and Other Structures.
ASTM E 1886-19. Standard Test Method for Performance of Exterior Windows, etc. impacted by Missile(s) and Exposed to Cyclic Pressure Differentials.
ASTM E 1996-23. Standard Specification for Performance of Exterior Windows, Curtain Walls, Doors and Storm Shutters Impacted by Windborne Debris in Hurricanes.
Cyclone Testing Station (CTS) 1978. Technical Report No. 440. Wind-borne debris impact tests.
Darwin Reconstruction Commission, 1975. Darwin Area Building Manual. Darwin Reconstruction Commission, Darwin.
Eckstein, D., Kunzel, V., Schafer, L., 2021. Global Climate Risk Index 2021: Who Suffers Most from Extreme Weather Events? Weather-Related Loss Events in 2019 and 200-2019. Germanwatch.
Florida Building Code (FBC) 2020. Florida Building Code Building. International Code Council (ICC).
Goliger, A.M., Milford, R.V., 1998. A review of worldwide occurrence of tornadoes. In: Journal of Wind Engineering and Industrial Aerodynamics 74-76 (1998), pp. 111-121.
Henderson, D., et al., 2018. Damage and loss to Australian engineered buildings during recent cyclones. International Workshop on Wind-Related Disasters and Mitigation Tohoku University, Sendai, Japan. March 11-14, 2018.
Herseth, A., Smith, T.L., Overcash, G., 2012. FEMA’s Coastal Construction Manual Update – Wind Resistant Design. In: Advances in Hurricane Engineering. Learning from Our Past. ASCE.
Intergovernmental Panel on Climate Change (IPCC), 2022. Climate Change 2022. Mitigation of Climate Change. Working Group III contribution to the WGIII Sixth Assessment Report of the Intergovernmental Panel on Climate Change.
International Building Code (IBC) 2021. International Building Code Building. International Code Council (ICC).
International Organization for Standardization 2018. Contributing to the UN Sustainable Development Goals with ISO standards. ISO Central Secretariat.
ISO/DIS 16316 “Windows, doors and curtain walling — Impacted by windborne debris in windstorms — Test method and classification.”
Kordi, B., 2009. Aerodynamics of windborne plate debris. Ph.D. thesis. Department of Civil and Environmental Engineering, Faculty of Engineering Science, University of Western Ontario.
Laboy, S., Dmith, D., Fernandez, G., Masters, F., 2012. Residential Fenestration Vulnerability to Windborne Debris. In: Advances in Hurricane Engineering: Learning from Our Past. ASCE, 2012.
Lu, S., X. Bai, X. Zhang, W. Li, and Y. Tang, 2019. The impact of climate change on the sustainable development of regional economy. In: Journal of Cleaner Production 233, pp. 1387-1395.
Mejorin, A., 2022. “Wind-Borne Debris Resistance of Façades: Identification of Alternative Impact Test Requirements”, Ph.D. thesis, Western University, London Ontario, Canada and Iuav University, Venice, Italy.
Munich RE, 2021. Record hurricane season and major wildfires – The natural disaster figures for 2020. Accessed on October 16th, 2022. https://www.munichre.com/en/company/media-relations/media- information-and-corporate-news/media-information/2021/2020-natural-disasters-balance.html.
Munich RE, 2022. Hurricanes, cold waves, tornadoes: Weather disasters in USA dominate natural disaster losses in 2021. Accessed on October 16th, 2022. https://www.munichre.com/en/company/media-relations/media-information-and-corporate-news/media-information/2022/natural-disaster-losses- 2021.html.
National Building Code of India 2016.
National Weather Service 1999. Accessed on March 1st, 2024: https://www.weather.gov/slc/SLC_Tornado# .
Piscitelli, F.M., Ruiz, J.J., Negri, P., Salio, P., 2022. A multiyear radar-based climatology of supercell thunderstorms in central-eastern Argentina. In: Atmospheric Research 277.
Scott D, Goupil J, Burton M, Denoon R, Larsen R, Spence SMJ, Wu T (2023) Advancement in Performance-Based Wind Design: Workshop Report. (National Institute of Standards and Technology, Gaithersburg, MD), NIST Grant Contractor Report (GCR) NIST GCR 23-045-upd1.
Tachikawa, M., 1983. Trajectories of flat plates in uniform flow with application to wind-generated missiles. In: Journal of Wind Engineering and Industrial Aerodynamics 14, pp. 443-453.
Testing Application Standard (TAS) 202-94. Criteria for Testing Products Subject to Cyclic Wind Pressure. Florida Building Code.
Testing Application Standard (TAS) 201-94. Impact Test Procedures. Florida Building Code.
World Economic Forum, 2022. The Global Risk Report 2022, 17th Edition. Insight Report, World Economic Forum.